I Introduction
Detecting gas molecules using semiconductor gas sensors is important for agriculture, chemical controls, environmental monitoring, and medical applications [1, 2]. Low-dimensional systems, especially material systems based on graphene, have for many years demonstrated outstanding developments in sensors and transistor applications [3{8]. However, since the band gap of graphene is almost equal to 0, it has not been fully exploited in the semiconductor industry [9]. The immense success of graphene [10-12] was followed by in-depth studies and encouraging eforts to nd other twodimensional (2D) nanostructures, such as silicene [13], phosphorene [14] single-layer graphitic zinc oxide [15], h-boron nitride [16], and transition-metal dichalcogenides [17]. Penta-graphene (PG), a novel wide band gap carbon allotrope, and PG-like materials were discovered in early 2015 [18-20]. It was found that PG is ultra-strong, and can sustain a temperature of 1000 K with grain boundaries. It displays a quasi-direct intrinsic band gap of 3.2 eV [18, 21]. Additionally, using the G0W0 approximation, the PG band gap was calculated as 4.2 eV [22]. In contrast to hydrogenated graphene, hydrogenation of PG leads to a notable increase (76% rise) in thermal conductivity instead of the 63% reduction expected due to heat dispersal in device operation [23]. Furthermore, PG is noteworthy due to its unique mechanical properties and anisotropic mechanical behavior [24]. Unlike graphene, PG has a buckle structure, which allows it to adsorb gas molecules in rich configurations. Thus, PG is considered an excellent base for the development of gas sensors which can detect harmful gases such as CO, CO2 and NH3 [25-27].
Cutting 2D-PG sheets in various crystallography directions obtains more diferent penta-graphene nanoribbons (PGNRs). Z. Q. Fan et al. found that the sawtooth-sawtooth PGNR (SSPGNR) is a more stable structure than the other three PGNR structures [28]. PGNR receives major attention in semiconductor material science since its energy band gap can be controlled efectively in many ways, such as by applying an electric eld and bending [29], doping [30, 31], and edge termination [32, 33]. In our previous studies [31] we discovered that the current intensity of N:SSPGNR increases to about 108 times that of pure SSPGNR. This is convenient for determining current strength in electronic devices, including sensors. Furthermore, we studied the adsorption feature of the gas molecules (CO, CO2 and NH3) on the SSPGNR surface [34]. The results confirm that SSPGNR is sensitive to CO and NH3 molecules, but less sensitive to the CO2 molecule [26, 27]. However, it is important to study the absorption configurations at the edges of nanoribbons [8,35]. SSPGNR can provide prior adsorption sites at its edges, which can serve as ideal gas sensor materials. Nitrogen doping at the carbon edge of PGNRs allows prior adsorption at this edge without hydrogen passivation. The carbon at the edge without hydogen passivation presents the dangling efect that favorably adsorbs CO, CO2 and NH3. In this study, using DFT and NEGF methods we investigate theoretically the electronic and transport properties of N:SSPGNR when gas molecules (CO, CO2 and NH3) are adsorbed on their edge.
The paper is organized as follows: the subject and research objectives are presented in the introduction section; in section II the computational methods are discussed; section III contains the results and discussion of the electronic and transport properties of N:SSPGNR with the adsorbed gas molecules on the edge; in section IV the conclusions are presented.
II Computational Methods
The electronic and transport properties of N:SSPGNRs which adsorb gas molecules were explored by rst-principles calculations based on DFT and NEGF, using the Atomistix ToolKit (ATK) software package (version 2017.1) [36, 37]. The width of the studied structure was six sawtooth chains. A 10 A vacuum space was introduced along non periodic (i.e., x and y) directions to ensure the isolation of N:SSPGNRs from their periodic replicas.
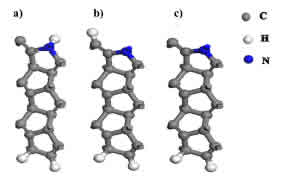
Figure 1: Schematic of possible N:SSPGNR adsor-bent sites for gas molecules, consisting of a) C-H- N:SSPGNR, b) H-N-N:SSPGNR, c) C-N-N:SSPGNR.

Figure 2: Adsorption configurations of C-H- N:SSPGNR consist of: a) C-H-N:SSPGNR, b) isolated gas molecules and c) configurations of C-H-N:SSPGNR after adsorption gas molecules.
The samples were optimized using DFT calculations within the generalized gradient approximation (GGA) of Perdew Burke Ernzerhof (PBE) [40], with the following similar conditions: 1 x 1 x 9 kpoint sampling, a cut-oenergy of 790 eV and electron temperature of 300 K. Considering the electric polarization eect, a double-zeta-polarized basis set was used to expand the electron wave function. The self-consistent eld tolerance was set as 10
III Results and Discussion i Structure Stability
To gauge the capacity of N:SSPGNRs to detect gas molecules (CO, CO2 and NH3), the adsorption of gas molecules was investigated on their edges, as the edge is the most reactive site on the ribbon due to the presence of dangling bonds.
Figure 1 depicts three possible adsorbent sites on the edge of an N:SSPGNR, including: a) removing a passive H atom at the top of the C atom (CH- N:SSPGNR), b) removing a passive H atom at the top of the N atom (H-N-N:SSPGNR), and c) removing both passive H atoms on the edge of the ribbon (C-N-N:SSPGNR). To explore the preferred conguration, we had to identify the model which the guest molecules would approach uninhibitedly. We in turn determined the most appropriate conguration for each gas molecule: CO, CO2 and NH3.
We rst considered possible adsorption configurations of the CO molecule on the edge of an N:SSPGNR; CO can be adsorbed vertically on the edge with the O atom either upward or downward. Therefore, there are 8 possible adsorption configurations with the CO molecule. Similarly, there are 12 possible adsorption configurations for the CO2 molecule and 8 for the NH3 molecule. These configurations are listed in Table 1. To determine the preferred configuration, we calculated the adsorption energy (Ead) of all the configurations considered, as follows [38, 39]:
where Etotal, Eribbon and Egas are the total energies of a considered configuration after gas molecule adsorption, removing a passive H atom nanoribbon and isolated gas molecules, respectively. As per the denition adopted here, negative adsorption energy shows that the process is exothermic in nature while the magnitude signies thermodynamic stability. Computed results indicated that COCH2, CO2CH2 and NH3CH2 (Fig. 2) were the preferred congurations of CO, CO2 and NH3 on N:SSPGNRs, respectively. The adsorption energy of these samples decreased gradually from -0.26 to -2.96 eV in the following order: Ead(NH3) > Ead(CO2) > Ead(CO). It is obvious that CO adsorption is the most stable.
In all three adsorption cases, the N:SSPGNR edge atom closest to gas molecules is a C atom rather than an N atom. This suggests that the most eective N:SSPGNR conguration for adsorption of gas molecules is the C-H-N:SSPGNR configuration. The adsorption distances of CO, CO2, and NH3 are 1.291 A, 1.487 A, and 3.317 A, respectively, as shown in Table 2. Therefore, CO- and CO2- adsorbed N:SSPGNRs are more capable of chemical adsorption than physical adsorption, while an NH3- adsorbed N:SSPGNR is more likely to adsorb physically [41].
ii Parameters of structures
Table 3 presents the parameters of three adsorption structures after relaxation. It can be clearly seen that the bond lengths of the gas molecules vary slightly. Namely, after the adsorption, the bond lengths of two adsorbed gas molecules (CO, CO2) are longer than those of isolated gas molecules. The opposite is true of NH3 gas molecules. In addition, the bond angle of the CO2 molecule is reduced from 180 to 122. The bond angles of the NH3 gas molecules change negligibly. In particular, the bond length of the CO adsorption sample changes the most. This is also one reason the adsorption energy in this sample is the largest. On the other hand, the bond lengths (d1, d2, d3) and the bond angles (1, 2, 3) close to the edge of C-H-N:SSPGNR substrate were also changed after the gas molecule adsorption. The changes in the structural parameters due to this interaction is the basis of the change in electronic properties, which will be analyzed in the next section.

Table 2: Adsorption energy (Ead), adsorption distance (h), band gap (Eg) and charge transfer (Q) from gas molecules to C-H-N:SSPGNR.
iii Electronic Properties
The electronic properties of C-H-N:SSPGNR were studied to understand its capacity to detect CO, CO2 and NH3. We rst investigated the density of states (DOS) of CO, CO2 and NH3-adsorbed C-HN: SSPGNR, as shown in Fig. 3. We also see that after the adsorption of gas molecules, the DOS of systems changed, and the band gaps of all three samples expanded. Specically, the band gap of CO-adsorbed C-H-N:SSPGNR showed the largest increase; its band gap increased to 2.41 eV while the band gap of C-H-N:SSPGNR was only 1.44 eV. On the other hand, the sample with the smallest band gap change was the NH3-adsorbed C-H-N:SSPGNR sample (Table 2). The trend seen in the calculated charge transfer (Q) in Table 2 can be understood as capacity for relative electron donation or electron withdrawal of the adsorbed molecules. The positive Q values mean that charge was transferred from the adsorbed molecules to the C-H-N:SSPGNR in all three cases, the charge transferred from the CO2 to the C-H-N:SSPGNR being the largest (Q = 0:47e).
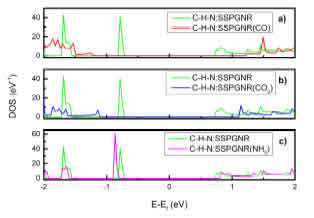
Table 3: Bond lengths of gas molecules before and after adsorption (l1 to l6), bond angles of gas molecules before and after adsorption (1 to 4), bond lengths at edge of C-H-N:SSPGNR before and after gas molecule adsorption (d1 to d4), and bond angles of C-H-N:SSPGNR before and after adsorption for gas molecules ( 1 to 4 ).
To better understand the cause of the changes in band gaps after adsorption, we analyzed the contribution of the gas molecules by drawing the total density of states (TDOS) and the local density of states (LDOS), shown in Fig. 4. We also see that the main contribution to the changes in DOS is not due to the gas molecules but to changes in the substrate. In particular, the CO molecule has the most in uence on DOS and the NH3 molecule has the least. In all cases, the major contribution to the changes in band gap width is the p orbitals of the atoms.
On the other hand, as can be see from Fig. 3 and Fig. 4, in all three cases there is an overlap between the DOS lines, which conrms the connection between the gas molecules and the substrate. However, in the case of CO and CO2 adsorption, there is an overlap and interlacing between TDOS and LDOS, so we can conrm that there is chemical bond formation. The adsorption in these two cases is chemical. In contrast, in the case of NH3 the adsorption is only the overlapping between TDOS and LDOS; NH3 can only physically adsorb on the edge of N:SSPGNR.
Figure 5 shows the electron density dierence (EDD) for all three adsorbed samples. The EDD was calculated using the following formula:
Here, (Total) and (ribbon) represent the total electron densities of the N:SSPGNR with and without adsorbed gas molecules, respectively, and (gas) is the electron density of the isolated gas molecules.
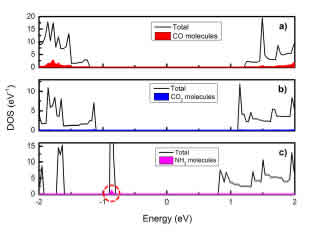
Figure 4: TDOS of systems: a) C-H-N:SSPGNR(CO); b) C-H-N:SSPGNR(CO2); c) C-H-N:SSPGNR(NH3) and LDOS of the gas molecules (the lled area under the DOS curve).
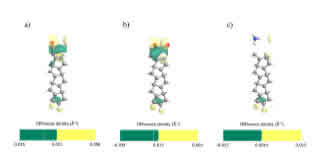
Figure 5: The electron density dierence for CO, CO2 and NH3-adsorbed N:SSPGNR. The isosurface value is taken as 0.009 eV A.
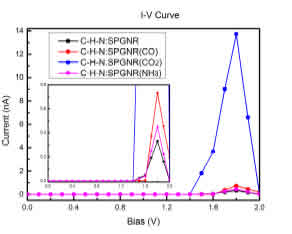
Figure 6: Calculated I-V curves for four structures: C-H-N:SSPGNR, C-H-N:SSPGNR(CO), C-H-N:SSPGNR(CO2) and C-H-N:SSPGNR(NH3
It can be seen from the EDD plots that the charges were accumulated over the adsorbed gas molecules. The formation of chemical bonds is evident in the case of CO and CO2 adsorption. The electron density at the interface region between CO, CO2 and N:SSPGNR indicates that the adsorbed gas molecule does form covalent bonds with the N:SSPGNR after the adsorption process. In contrast, in the case of NH3 adsorption, no formation of covalent bonds was found, because there is little electron density di erence at the interface between the NH3 gas molecule and N:SSPGNR.
iv Transport Properties
In the previous section, we showed that the adsorption of gas molecules causes changes in the electronic band gaps of N:SSPGNR. For further verification of CO, CO2 and NH3 detection by N:SSPGNR, we studied the transport properties of C-H-N:SSPGNR before and after the adsorption of gas molecules. The current voltage (I-V) characteristics were obtained using a two-probe model. Figure 6 shows the correlation between the current and the bias voltage of the adsorbed samples and the pure sample. We can see that all of these lines have the same shape. On the graph there is a conduction pause although the bias voltage increases. When the bias voltage reaches a certain limit value (1:6 V), the current begins to increase, then decreases until saturation at 2:0 V. Specifically, except for the CO2 adsorption sample, the current of the remaining samples starts to increase at the bias voltage of 1.6 V (threshold bias). For semiconductors, when the polarizing voltage is not large enough the device stops conducting, but when it is large enough for an electron to cross the barrier, the device begins to conduct electricity. Thus, we can conclude that the structures under consideration are semiconductors. The maximum current obtained for pure C-H-N:SSPGNR, CO-adsorbed C-H-N:SSPGNR, CO2-adsorbed C-H-N:SSPGNR, and NH3-adsorbed C-H-N:SSPGNR is 0.3 nA, 0.7 nA, 13.7 nA, and 0.4 nA, respectively. In all four cases, the maximum currents occur at the bias voltage of 1.8 V. The maximum current of the CO2 adsorption sample is the highest, 45 times greater than that of the pure sample. The maximum current of the NH3 adsorption sample is the lowest, only 1.3 times greater than that of the pure sample. All these ndings suggest that the N:SSPGNR current can be distinguished before and after molecule adsorption [42].
To explain the change in the trend of the I-V curves in Fig. 6, we considered the bias dependent transmission spectra, T(E,Vb), of the four studied structures via Fig. 7 (left-hand panels). We see that the T(E,Vb) of the pure C-H-N:SSPGNR, the CO-adsorbed C-H-N:SSPGNR, and the NH3- adsorbed C-H-N:SSPGNR difer very little. However, the T(E,Vb) spectrum of the CO2-adsorbed C-H-N:SSPGNR is very diferent from the three other samples. This is also related to the charge transfer phenomenon mentioned in Table 2. Furthermore, the various maximum currents occur at the bias voltage of 1.8 V; the transmission coeficients at 1.8 V bias of the four structures were calculated and shown in the right-hand panels of Fig. 7. The values and lled zones bounded by the horizontal axis and T(E, Vb = 1.8 V) curves help us to explain the changing tendency of the maximum currents of the four structures.
IV Conclusions
In summary, using rst-principle calculations we studied the adsorption geometry, adsorption energy, charge transfer, density of states, partial density of states and I-V curves of N:SSPGNR with gas molecule (CO, CO2 and NH3) adsorption. Our calculated results show that edge adsorption of CO and CO2 molecules is more energetically favorable than edge adsorption of NH3. Moreover, the adsorption of CO and CO2 on the edge of N:SSPGNR is chemical, while the adsorption of NH3 is physical. The current voltage (I{V) characteristics were also investigated using the non-equilibrium Green's function (NEGF) approach. The results indicate that conductance of the CO, CO2, and NH3 adsorption N:SSPGNR can be distinguished at the bias voltage of 1.8 V. These changes in electronic and transport properties make N:SSPGNR a promising candidate for gas detector development.
Acknowledgements
This research was funded in part by the Can Tho University Improvement Project VN14-P6, supported by a Japanese ODA loan. We are grateful to the information center and network administrator of Can Tho University (CTU) for computational support. We also thank Prof. Yoshitada Morikawa (Osaka University) for discussing research ideas.