Introduction
Through millions of years of evolution, reptiles have developed a peculiar heterogeneity of reproductive patterns and, consequently, strategies necessary to adapt to the land habitat (Reisz, 1997). The amniote egg represents an outstanding innovation that has linked the composition and conformation of the shell with metabolism, development, and embryonic survival (Hallmann and Griebeler, 2015). This adaptation against desiccation implies that the egg must contain all the necessary components to guarantee extrauterine development (Schmidt-Nielsen, 1997). To ensure this dynamic challenge, the shell controls the exchange of water and gases, protects the embryo from microbial attack, and is a source of minerals (Osborne and Thompson, 2005; Chang and Chen, 2016).
Considering the structural organization of the eggshell and the sensitivity to the hydric environment of the nest, it is possible to distinguish eggs with strongly calcified eggshells, characteristic of some gekkotan species (Schleich and Kästle, 1988; Pike et al., 2012) and eggs with flexible shell or parchment-shelled eggs, distinctive of lizards and snakes (Packard et al., 1982b; Hirsch, 1983).
Recently it was demonstrated that the eggshell of Salvator merianae has an organic and inorganic composition notably different from that described for the parchment-shelled eggs of reptiles (Campos-Casal et al., 2020). It was found the presence of hydroxyapatite in the deep section of the shell, compact and alveolar fibers, and although it was not characterized at the molecular level, the histological analysis revealed a glycoprotein coating on the outer surface of the egg (Campos-Casal et al., 2020).
Eggshells are a perfect example of the organic-inorganic concept made up of multifunctional biopolymers and biominerals, recognized for their lightweight, strong, and resistant structure (Fratzl and Weinkamer, 2007). The remarkable toughness and damage tolerance of these biological materials are conferred through the hierarchical assembly of their architectures, and multiscale components (Weinkamer and Fratzl, 2011). The organic components that makeup biopolymers are protein-forming polypeptides (collagen, keratin, elastin, resilin, fibroin, abductin), and glycosaminoglycans ENT#091;GAGs; (Chen et al., 2012; Scott and Panitch, 2013)ENT#093;.
GAGs are a small family of polymers consisting of unbranched chains of repeated disaccharide units containing hexosamine, and hexuronic acid or hexose (Ellis et al., 2009). Because of their tendency to occupy large domains in aqueous solution, GAGs are responsible for the structural and mechanical functions of the extracellular matrix ENT#091;ECM; (Raman et al., 2005)ENT#093;.
There are two major types of GAGs. Sulfated GAGs include chondroitin sulfate, dermatan sulfate, keratan sulfate, heparin, and heparan sulfate; while hyaluronic acid (HA) or hyaluronan is the only non-sulfated GAG (Gandhi and Mancera, 2008). HA is a biopolymer composed of a disaccharide sequence of N-acetylglucosamine (GlcNAc) and D-glucuronic acid (GlcA) linked by alternating glycosidic bonds β-1→4, and β-1→3 (Nusgens, 2010). Due to the high number of carboxyl and hydroxyl groups, HA is a highly hydrophilic biomaterial, that forms a gel-like structure in aqueous solution as a result of intermolecular interaction among linear polymers (Zhu et al., 2017). In terms of water transport, diffusion, and ion exchange, the physiological functions of tissues are determined by the concentration of HA and its molecular weight (Dicker et al., 2014). Besides, this polymer has shown to possess significant bacteriostatic properties (Romanò et al., 2017; Chen et al., 2019). The remarkable viscoelastic and water retention properties of HA, in addition to its biocompatibility, biodegradability, and null immunogenicity, have amplified the attractiveness of this biomolecule in the field of tissue engineering (Fallacara et al., 2018).
The study of bird eggshells has been a focus of interest in the emerging field of biomaterials (Baláž, 2014; Sah et al., 2016) for its content of GAGs (Liu et al., 2014), collagen fibers (Zhao and Chi, 2009), and keratins (Nys et al., 2001). Particularly high proportions of HA have been shown in the calcified fraction in the eggs of this animal group (Heaney and Robinson, 1976; Liu et al., 2014).
Although reptile eggshells have been extensively studied, the focus has been on the general morphology of their structure, and on the mechanisms of mineralization (Kusuda et al., 2013; Hallmann and Griebeler, 2015). However, at present, there is insufficient information available in terms of structural characterization at the multi-scale level, compositional analysis, and mechanical properties associated with the biomaterials that make up the eggshell of reptiles (Chang and Chen, 2016).
In this context, investigating and identifying the constituent biopolymers of S. merianae eggshells would offer alternative designs for the multiscale study of new multifunctional biological materials. The structural particularities, chemical composition (Campos-Casal et al., 2020), and high hydric requirements of the S. merianae egg (Manes, 2016), establish criteria for investigating the molecular nature of the glycoprotein coating of the eggshell. In perspective, knowing the conformation of this physiological structure, and the behavior of its structural components would allow establishing application parameters for the development of artificial incubation technologies.
In the present work, we analyze the ultrastructure of the surface of the recently oviposited egg from S. merianae with transmission electron microscopy (TEM), we examine the superficial glycosidic component with optical microscopy and we determine the molecular fingerprint of the glycoprotein covering using Raman spectroscopy.
Materials and methods
Biological material
To exclude possible modifications in the structure and chemical composition of the shells associated with incubation or embryonic development, eggs recently oviposited in a perfect preservation status of conservation were used. The eggs (n = 24) were taken from six nests built by S. merianae females (with at least two previous egg-layings). The average weight of the females was 4 kg, and the average snout-vent length > 35 . The animals were housed in the experimental lizard farm of the Facultad de Agronomía y Zootecnia of the Universidad Nacional de Tucumán, located at Finca El Manantial (26º 51’ S, 65º 17’ W), province of Tucumán, Argentina. The protocols for the handling and care of the animals were carried out by the “Guide for the care and use of laboratory animals” (Committee for the update of the guide for the care and use of laboratory animals, 2011). All experiments performed were approved by the ethics committee of the Research Council of the Universidad Nacional de Tucumán (CIUNT).
Optical microscopy
For the histochemical studies, 12 whole eggs were fixed in 4% formaldehyde solution in phosphate-buffered saline at pH 6.8 (Suvarna et al., 2008) for 24 h at 4º C. Small pieces of shells from each egg (n = 24) were dehydrated in ethyl alcohol, diaphanized in xylene, included in paraffin-celloidin and serially sectioned at 8 μm.
For the determination of the GAGs, the sections were stained for 15 minutes in a solution of 1% Alcian Blue 8GX (AB; Sigma-Aldrich, Buenos Aires, Argentina) pH 2.5 (Suvarna et al., 2008). After washing in distilled water, the shell samples were stained with a Periodic Acid-Schiff (PAS) kit (Biopur, Rosario, Argentina). The sections were then dehydrated in absolute ethyl alcohol and mounted in acrylic resin (Biopack, Buenos Aires, Argentina).
The samples were photographed with an Olympus BH2 Microscope (Olympus Corporation, Tokyo, Japan) equipped with a Canon Eos Rebel T3i digital camera (Canon Corporation, Tokyo, Japan).
Electronic microscopy
Small pieces of eggshells (n = 12) were fixed in half-concentration Karnovsky´s fixative ENT#091;2.5 % glutaraldehyde, 2% formaldehyde, pH 7.4; (Karnovsky, 1965)ENT#093; for 24 h at 4-5 ºC, post-fixed with 2% osmium tetroxide (Ted Pella, California, U.S.A), dehydrated in increasing concentrations of acetone and included in Spurr resin (Ted Pella, California, U.S.A). The ultrasections were contrasted with 2% Uranyl Acetate (Ted Pella, California, U.S.A) and observed with Zeiss Libra 120 Transmission Electron Microscope (Carl Zeiss, Oberkochen, Germany) controlled with Win TEM user interface and system software (Carl Zeiss).
Raman spectroscopy and statistics
The spectroscopic examination was performed with a confocal Raman Microscope DXR (Thermo Fisher Scientific, Waltham, Massachusetts, USA) equipped with an excitation wavelength of 780 nm at 24 mW of power (5 -1 spectral resolution). A confocal aperture of 50 μm was used for data collection. The samples were focused with a 20x objective. All measurements were made at room temperature.
The outer surface of the recently oviposited egg of S. merianae exhibits irregular plaques separated by fissures (Campos-Casal et al., 2020). This configuration was considered to establish the five consecutive sampling points on the shell surface (n = 6). Indeed, from left to right ( 3C), points one, two, four, and five correspond to the samples taken in two consecutive plaques. Point three was sampled in the center of a fissure ( 3C). Each sampling point produced an individual spectrum, which was acquired by accumulating 100 exposures with an exposure time of 5 s each.
A total of 30 spectra were measured from the samples. The spectral profile of most of the sampled points proved to be very similar to each other, allowing the generation of a single average-spectrum representative of the overall spectral behavior of the plaques and fissures. The spectral adding and baseline correction of the average-spectrum were performed with the optical spectroscopy software Spectragryph. The overlapping frequencies were mathematically decomposed with the Origin Pro software, using the Voigt function.
The statistical analysis was performed with In foStat software (Di Rienzo et al., 2018). The t-test was used to compare the HA distribution on the plaques and the fissures using the vibrational frequencies and the Raman intensities of the average-spectrum.
Results and discussion
Optical microscopy and surface ultrastructure
Microscopic examination of the AB pH 2.5 and PAS stained sections exhibited an intense GAGs coating on the outer surface of the S. merianae eggshell (Figure 1).
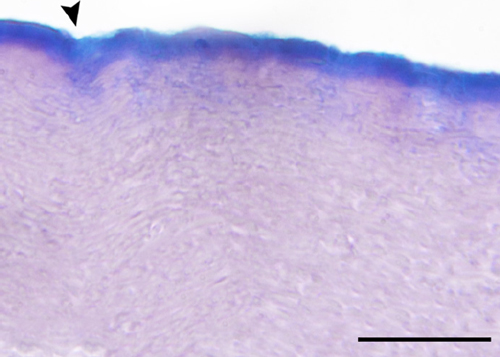
Figure 1 Light micrograph of a cross-section through eggshell stained with AB pH 2.5 and PAS showing a continuous coating of glycoproteins on the outer surface. The arrowhead points to a fissure. Scale bar 30 µm.
Ultrastructural analysis showed two different regions, recognizable by their amorphous appearance and fibrillar content (Figures 2A and 2B). Thus, the outermost section forms a continuous cover composed of different electrodense zones (Figures 2A and 2B). In particular, under the free surface of the shell, a band with moderate electronic density was observed (Figure 2B). Below this outer cover, small alveolar fibers (Figure 2A) were observed included in a matrix with a granular configuration (Campos-Casal et al., 2020). However, crystalline structures were not recognized, a characteristic incompatible with the idea that supports the presence of calcium carbonate in soft and hard shells of reptiles (Packard and DeMarco, 1991; Choi et al., 2018). It has been shown that the only biomineral present in the eggshell of S. merianae is hydroxyapatite, located in the deep section of the eggshell (Campos-Casal et al., 2020).
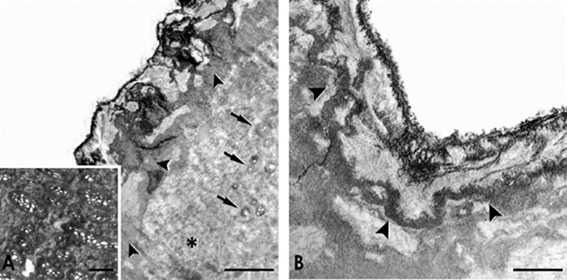
Figure 2 TEM photographs of the eggshell surface of S. merianae. A) Note the electrodense zones (arrowheads) and the granular matrix (asterisk) with small alveolar fibers cross-sectioned (arrows). The insert shows superficial alveolar fibers surrounded by interfibrillar material. Scale bar 2 µm. B) Detail of the moderate electron density band (arrowheads) underlying the surface of the shell. Scale Bar 1 µm. In both images, the outside of the eggshell is on top.
Raman spectroscopy
Raman spectroscopy has proven to be an outstanding tool for the structural characterization of glycosaminoglycans (Arboleda and Loppnow, 2000). Raman frequencies assignments for HA and its monomeric components have generally been reported using purified biomolecules (Reineck et al., 2003; Meziane-Tani et al., 2006; Synytsya et al., 2011). However, in complex biological systems, the Raman spectrum of numerous biopolymers may exhibit shifts in the position of their frequencies (± 1-5 -1) as a result of structural and conformational alterations (Bergholt et al., 2016).
The analysis of the average Raman spectra of plaques and fissures of the eggshell of S. merianae are shown in Figures 3A and 3B. The main characteristic bands of the HA were determined in two spectral windows: 600-1,800 -1 (Figure 3A), and 2,800-3,000 -1 (Figure 3B). The Amide I (AmI) and Amide III (AmIII) domains of the HA can be easily distinguished along with bands associated with carbohydrate chains and several amino acid vibrations.
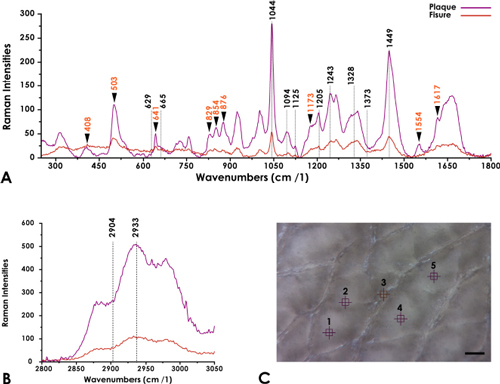
Figure 3 Average-spectrum of plaques and fissures. A) Representative Raman average-spectra between 300-1,800 cm-1. The graph indicates the tentative assignments of the HA in plaques and fissures. Amino acid frequencies are indicated with an arrowhead. B) Representative Raman average-spectra between 2,800-3,000 cm -1. C) Microphotography of the outer surface of the eggshell indicating the five sampling points corresponding to average-spectra in images A and B. Scale Bar 100 µm.
The spectral section between 610-683 -1 contains bands assigned to the out-of-plane vibration modes of the groups CCO, CCH, C=O, and CO. Although these vibrations have been assigned to HA (Synytsya et al., 2011), the Raman spectral analysis of the GlcA suggested that the bands at 629 -1 and 665 -1 would be distinctive of this constituent monomer of HA (Meziane-Tani et al., 2006).
The deconvolution of the spectral range between 850-980 -1 (Figures 4A and 4B) evidenced in the average-spectrum of the plaques and the fissures the overlapping of frequencies at 864 -1, 899 -1, 923 -1, 951 -1, 960 -1, and 970 -1, vibrations typical of the bonds of the anomeric skeletal configuration (α or β), and the glycosidic bonds (Yuen et al., 2009). These frequencies are similar to those reported for HA (Bansil et al., 1978; Barret and Peticolas, 1979).
In general, the main spectral features of carbohydrate chains are associated with C-O stretching coupled with C-O-H deformation modes, which can be observed as a triplet of peaks at 1,025-1,045 -1; 1,080-1,100 -1 and 1,110-1,152 -1 respectively ENT#091;Figure 3A; (Bansil et al., 1978; Katsumata et al., 1996)ENT#093;. In particular, the region covering the range of 1,000 -1 and 1,100 -1 was associated with the vibrations of the saccharide molecular bonds that correlate with the C-O-C, C-C-C, and C-C-O stretching of the pyranose ring of the GAGs (Parker, 1983). Our spectra exhibited bands of C-C and C-O stretching vibrations at 1,044 cm-1, bending vibration of C-OH from the acetyl group (~1,094 cm-1) and a peak formed by bending vibrations of C-OH and C-H (1,125 cm-1). In particular, the frequency ~1,094 cm-1 has been assigned to the symmetric and antisymmetric vibrations of the β-glycosidic bonds (Sekkal et al., 1995; Alkrad et al., 2003) of the HA. Besides, the ~1,125 cm-1 band is considered an outstanding marker for the determination of this biopolymer, considering that this vibration is exclusive of glucose derivatives (Bansil et al., 1978). A medium intensity peak, representative of bending vibrations of CH2 at 1,205 cm-1, was also determined in both average spectra.
On the other hand, it was possible to establish a second spectral region (Fig. 3A) corresponding to the AmIII complex (1,215-1,350 cm-1). In effect, in this vibrational segment, bands were determined at 1,243 cm-1 belonging to the irregular or disordered domains (random coils), and at 1,328 cm-1 features of the saccharide moiety reported for the N-acetyl-monosaccharides (Bansil et al., 1978; Oleinikov et al., 1999). On the other hand, the vibrations representative of the asymmetric and symmetric bending of the C-H3 groups of the HA were distinguished at 1,373 cm-1 and 1,449 cm-1 respectively (Kotzianova et al., 2015).
The broad AmI spectral region between 1,600 cm-1 and 1,700 cm-1 (Kitagawa and Hirota, 2006; Rygula et al., 2013), observed in our spectra, consists of overlapping vibrations related to carbonyl group stretching reported for HA (Kotzianova et al., 2015). The spectral deconvolution of this region (Figures 5 A and B) in both average-spectra of the eggshell revealed vibrations at 1,600 cm-1, 1,630 cm-1, 1,636 cm-1, 1,655 cm-1 and 1,664 cm-1 respectively. In particular, the frequencies of the shoulders at ~1,600 cm-1 and ~1,630 cm-1, have been suggested as representative of the asymmetric COO- stretching of the HA (Synystsya et al., 2011; Essendoubi et al., 2016). Also, the band at 1,655 cm-1 would distinguish the vibrations of the C=C groups and the C=O groups of AmI of this biopolymer (Synystsya et al., 2011).
The second spectral window analyzed between 2,800-3,000 cm-1 (Figure 3B), contains two bands at 2,904 cm-1 and 2,933 cm-1 respectively. Indeed, these vibrations represent the C-H and N-H stretches of the HA (Donghui et al., 2006; Essendoubi et al., 2016).
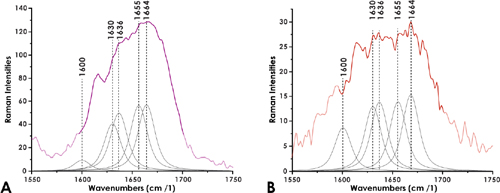
Figure 5 Decomposition of the Raman spectra of plaques (A) and fissures (B) corresponding to the vibration of the AmI complex.
In addition to the characterization of the amides bands of HA, there are additional characteristics in the Raman spectra that allow the description of the protein environment as a function of the amino acid side chains (Rygula et al., 2013).
Indeed, Figure 3A shows a prominent Raman band ~503 cm-1, which can be assigned to the S-S stretching mode of cysteine (Cys) and cystine residues (Widjaja and Garland, 2010). This vibration confirms that the conformation of the C-C-S-S-C group is gauche-gauche-gauche, largely stable conformation (Edwards et al., 1998). Two other pure components vibrations associated with the C-S stretching of Cys and cystine residues are resolved with a prominent band at 641 cm-1 and a shoulder at ~663 cm-1 respectively (Akhtar and Edwars, 1997; Widjaja and Garland, 2010). In our spectra, an overlap of the ~663 cm-1 band with the frequency at 665 cm-1 of the HA could be observed.
On the other hand, the characteristic Fermi doublet of the tyrosine (Tyr) is distinguished at 829/854 cm-1, along with two additional vibrations at 1,173 cm-1 and 1,617 cm-1 (Zhu et al., 2011). Finally, the characteristic vibrations of proline (Pro) and hydroxyproline (Hyp) were recognized at 408 cm-1, 876 cm-1, and 1,554 cm-1 respectively (Zhu et al., 2011).
The comparison of the relative intensities of the HA bands in the average-spectrum of the plaques and the fissures using the t-test indicates the absence of significant differences (P = 0.4308) in the distribution of HA and provides additional evidence to the histochemical studies, confirming that this biopolymer forms a continuous biofilm on the outer surface of the eggshell of S. merianae.
Many of the physiological effects of HA are related to its molecular weight (Hascall et al., 2004; Medina et al., 2006). Indeed, the HA of low molecular weight stimulates gene expression in macrophages, chondrocytes and some epithelial cells (Euppayo et al., 2015; Jariyal et al., 2020). On the contrary, the HA of high molecular weight characteristic for its viscoelastic properties (Gřundělová et al., 2015) modulates the hydration of tissues, the osmotic balance (Salwowska et al., 2016) and organizes the ECM (Manou et al., 2019).
Raman spectroscopic studies of the HA with different molecular weights showed that the intense peak observed at ~1,661 cm-1 in the HA of 1,200 kDa reveals a remarkable widening and reduction in the spectral intensity compared to the HA of 31 kDa (Alkrad et al., 2003). By analogy, the band at 1,664 cm -1 in the average-spectrum of the plaques and the fissures, would be similar to the vibrational profile of the HA of high molecular weight described in those studies. Although the results presented here do not offer information related to the molecular weight of HA on the outer surface of the eggshell of S. merianae, it is remarkable that the vibrational frequency in our spectra at 1,243 cm-1 and 1,664 cm-1 are characteristic of the irregular or disordered domains of the AmIII and AmI complex respectively (Rizo et al., 2016). Although being a linear molecule, in aqueous solution, the HA of medium and high molecular weight show an expanded random coil conformation (Chakrabarti and Park, 1980; Scott et al., 1991) optimal formation that provides local rigidity and high viscosity (Ingr et al., 2017). Also, the size of the random coils can be modified by the pH and the concentration of salts in the medium, characteristics of a flexible polyelectrolyte (Lapĉik et al., 1998), capable of bridging transient hydrogens with water molecules (Almond, 2005; Blundell et al., 2006). In vivo studies using Raman spectrography have shown that high molecular weight HA applied to skin samples remains on the surface forming a film that prevents water loss by evaporation (Essendoubi et al., 2016).
Several polymers studied as antibacterial coatings, HA shows a proven ability to produce biofilms capable of reducing bacterial adhesion (Romanò et al., 2017). Research related to the bacteriostatic effect of HA showed that this biopolymer with high molecular weight presents significant antiadhesive and inhibiting properties of bacterial growth (Pirnazar et al., 1999; Chen et al., 2019). Similar results were obtained by Carlson et al. (2004) comparing the bacteriostatic properties of HA with collagen Type I and hydroxyapatite, materials widely used in the manufacture of biomatrixes.
Considering the high sensitivity of the S. merianae egg to variations in the hydric environment of the nest and the absence of a calcareous coating (Campos-Casal et al., 2020), the HA cover in a hydrated environment would create a viscoelastic hydrogel that also to preserve the developing embryo against desiccation, it would participate in the protection, lubrication, and stabilization of the shell surface. Besides, due to its high molecular weight and its rheological properties, HA would form a stable biofilm, with characteristics of a semi-plastic fluid (Sudha and Rose, 2014), adaptable to the volumetric changes experimented by eggs with a flexible shell during incubation (Andrews, 1997). As we have indicated, studies related to the chemical composition of the S. merianae egg revealed a band of hydroxyapatite located in the deep zone of the shell (Campos-Casal et al., 2020). Thus, considering the physicochemical characteristics of the incubation environment (Manes, 2016), it would be fair to consider that HA and hydroxyapatite could work synergistically to protect the egg against bacterial invasion. The presence of HA as a bioconstituent in reptile eggshell has not been previously reported. However, in birds, this biopolymer is abundant in the shell membranes, and the calcified matrix (Liu et al., 2014; Vuong et al., 2017).
Sexton et al. (2005) compared the amino acid distribution of eggshells from 24 lizard species, 6 snake species, and 4 external groups, including birds eggshells, and determined that eggs with flexible shells, such as S. merianae, contain significantly higher levels of Pro and Tyr compared to rigid shells. As we have shown, the vibrational frequencies of both amino acids are present in the eggshell of S. merianae. Significantly, collagen contains high proportions of Hyp, Pro and glycine (Sorushanova et al., 2019), a biopolymer which in interaction with GAGs gives the distinctive mechanical resilience of the ECM (Holmes et al., 2018).
Studies of the structure and mechanical properties of the Taiwan cobra snake eggshells (Naja atra) showed that the flexible eggshells of this reptile behave like a highly extensible elastomer; quality determined by the presence of keratin and collagen fibers (Chang and Chen, 2016). Likewise, collagen I, V, and X have been characterized in the eggshell of birds (Arias et al., 1991).
Scanning electron microscopy analysis showed that the S. merianae eggshell is made up mostly of alveolar fibers, and a double layer of compact fibers, both immersed in an amorphous interfibrillar matrix (Campos-Casal et al., 2020). On the other hand, the elemental composition of the S. merianae eggshell using energy-dispersive X-ray spectroscopy determined a high sulfur content in the surface layer (Campos-Casal et al., 2020), higher than that reported for eggs of other reptiles with calcified shells (Choi et al., 2018). Although the ultrastructural studies only provide morphological evidence, it is reasonable to consider that the Pro and Hyp amino acid residues determined in the Raman spectra in the present work are representative of collagen fibers. In complex biological systems such as ECM, HA provides mechanical stability to collagen fibers (Wang et al., 2010). On the other hand, considering that sulfur is usually bound to proteins associated with keratin (Rogers et al., 2006), sulfur amino acids such as Cys and its dimer characterized in our spectra, offer consistent evidence to consider keratin and/or sulfated GAGs as constitutive biopolymers of the S. merianae eggshell.
A hydrophilic HA covering and protective viscoelastic on the outer surface of the S. merianae eggshell exposes a new perspective to establish correlations between the conditions of humidity and incubation temperature. Indeed, both physical parameters are substantial to develop artificial incubation technologies with a productive impact. On the other hand, the results presented here provide complementary evidence on the adaptations by which reptile eggs and embryos control the exchange of water with the incubation environment.
Conclusions
For the first time, an HA coating is identified in the eggshell of a reptile. The qualities of HA to maintain conformational rigidity and hydrophilicity; added to the ability to absorb impacts and interact with other GAGs and fibers of the ECM make it an important biomaterial. Therefore, the eggshell of S. merianae could offer an innovative platform for the study of structural, extensible, and lightweight biomaterials.